Location:Home > News >
FIBER OPTIC TECHNOLOGY AND ITS ROLE IN THE INFORMATION REVOL
INTRODUCTION
Everywhere on this planet hair-thin optical fibers carry vast quantities of information from place to place. There are many desirable properties of optical fibers for carrying this information. They have enormous information-carrying capacity, are low cost, and possess immunity from the many disturbances that can afflict electrical wires and wireless communication links. The superiority of optical fibers for carrying information from place to place is leading to their rapidly replacing older technologies. Optical fibers have played a key role in making possible the extraordinary growth in world-wide communications that has occurred in the last 25 years, and are vital in enabling the proliferating use of the Internet.
Of key importance in the course of these developments in information technology have been a few basic, but vitally significant, events. Principal among these are the invention and development of the laser, the growing appreciation that this might make optical communications practically useful, the production of very pure glass, which was sufficiently transparent that long distance transmission of light through glass fibers became practical, and the digital revolution. We will examine the role that each of these has played in creating the "Information Age."
THE LASER
Although the physical principles underlying the laser were first explained by Albert Einstein early in this century, it was not until the Spring of 1960 that Theodore Maiman, working at the Hughes Research Laboratories in Malibu, California, produced the first operating laser. This laser, which used a special ruby crystal, produced intense flashes of red light, a light with properties quite different from that emitted by conventional sources. A laser emits light in a very narrow beam– much like a searchlight. Laser light is very spectrally pure -- it is of a very pure, well-defined color -- and is very bright. These properties make a laser ideal for shining light from place to place over long distances, and it was soon proposed that this provided new opportunities to expand the usefulness of optical communication links.
Over the last almost forty years, developments in the laser field have occurred at a rapid pace. Many new lasers have been discovered, each with its own special properties and applications, and their cost, performance, and practicality have all shown dramatic improvement. Although the laser was first described as "a solution looking for a problem," this is no longer the case. These devices affect our lives in many ways, every day. At the supermarket checkout a laser illuminates the bar code on each purchase, allowing its rapid identification and pricing. Every compact disk player contains a low-cost, semiconductor laser, whose beam illuminates the pattern of information encoded into the surface of the CD and allows its rapid re-translation into music or computer data. At the doctor’s office, or in hospitals, lasers are used for many minor and major surgical procedures: removal of skin cancer, birth marks, and other lesions, for the fragmentation of kidney stones, the welding back into place of detached retinas, for cosmetic surgery, photodynamic therapy, and as a specialized scalpel.
The most widely used type of laser is the semiconductor laser, which has much in its technology in common with the semiconductor devices that we use in modern electronic devices. The principal difference between these two semiconductor technologies is that conventional electronic semiconductors are almost exclusively based on the element silicon, whereas semiconductors used in lasers (and related so-called optoelectronic devices) are based primarily on mixed semiconductors made up from gallium (Ga) and arsenic (As) , and often aluminum (Al) as well. It is semiconductor lasers made up from GaAs/GaAlAs that provide the light for optical communication along optical fibers.
OPTICAL COMMUNICATIONS
Optical communications systems have a long history. Ancient man signalled with smoke and fire, often relaying messages from mountain top to mountain top. However, this optical communication scheme had limited transmission capacity. They could serve as a warning, as Queen Elizabeth the First of England planned when she had a network of bonfires erected to be set in the event of a seaborne invasion from Spain. The smoke signals transmitted by native Americans had the capacity to transmit various messages. Since the end of the eighteenth century messages have been passed by semaphore – the use of flags to indicate the transmission of one letter at a time. This form of communication could transmit information at a rate of about one letter per second over a direct line of sight, although messages could be relayed over long distances. Such means of communication were not very secure: anyone in the line of sight to the message sender could read the information (if he knew the code). The message could also be intercepted and altered during the relay process as the Count of Monte Cristo did to his advantage .
Another historical use of optical communication involved the heliograph -- a device to reflect the sun's rays from a transmitting to receiving station using a code. This technique was widely used by the US Cavalry in the desert south-west of the United States until the early part of the twentieth century. For optical communication to progress past these early efforts, an information carrying channel had to be developed that was reliable, inexpensive, and that could be used over long distances, preferably at high rates of data transmission. The fundamental physical phenomenon that makes this possible is called total internal reflection. This phenomenon causes light to reflect, rather than refract, when it attempts to cross the boundary from one transparent optical medium to another of lower optical density, at a sufficiently large angle. As early as 1854, in London, John Tyndall demonstrated that light could be guided inside a transparent medium with such a density discontinuity with its surroundings. He did this by showing light being guided along a stream of water flowing from a container. His simple demonstration proves that in the right circumstances light need not travel in straight lines.
TOTAL INTERNAL REFLECTION
When a ray of light passes from one transparent medium to another, for example at the surface of a pool of water, it generally bends at the boundary. This phenomenon is well known: a stick poked into water appears to bend. The bending of the ray at the boundary is described by Snell’s law, a simple relationship between the sines of the angles that the ray makes on the two sides of the boundary. Mathematically, Snell’s law can be written as:
n1sinq 1=n2sinq 2
where q 1 is the angle of the ray on one side of the boundary, as shown in Fig.(1), and q 2 is the angle on the other side. The quantities n1 and n2 are the refractive indices of the media on opposite sides of the boundary.
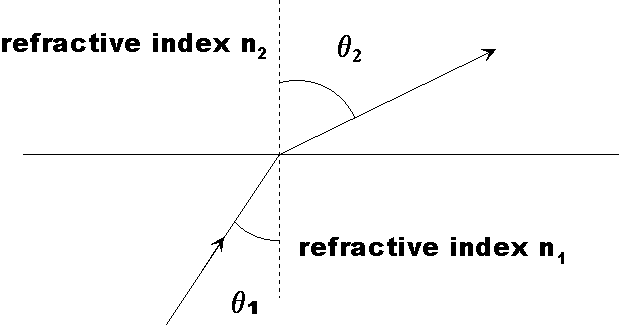
The refractive index of each medium is a number that characterizes the optical density of the medium relative to a vacuum. It is a number that describes how much more slowly light travels in the medium relative to its velocity in a vacuum. If n2 is less than n1 this equation restricts the angles at which a ray of light can get across the boundary. If light is passing from medium 1 to medium 2 and the angle q 1 is greater than the critical angle, then the light can not refract across the boundary (because q 1 can not be greater than 90o). The critical angle is the angle whose sine is n2/n1. When a ray of light strikes a boundary at an angle greater than the critical angle it reflects, and does not cross. Although many devices that we use in real life use this phenomenon, and although many natural optical phenomena depend on its occurrence, we may not be aware of it. A simple example can be given, which involves what a swimmer sees who is underwater and attempting to look upwards through the surface. If such a swimmer looks more or less straight up towards the surface, he or she will be able to see what is above the surface. On the other hand if he or she looks at a sufficiently large angle towards the surface, only a reflection of whatever is below the surface will be seen. Of course, a fish would experience the same effect and can only see over a range of angles near the perpendicular to the surface. This phenomenon is of real practical significance to fisherman, because a fish seeing an attractive lure can not see the rod to which it is attached, nor the fisherman.
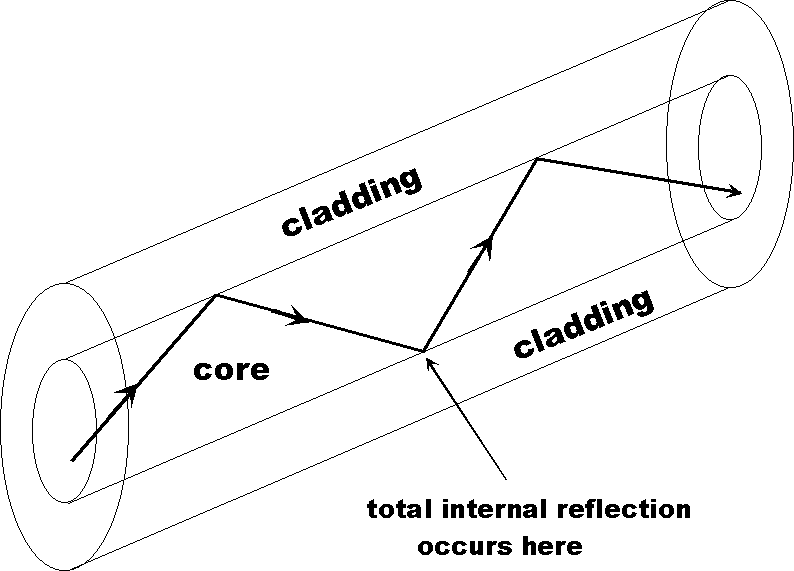
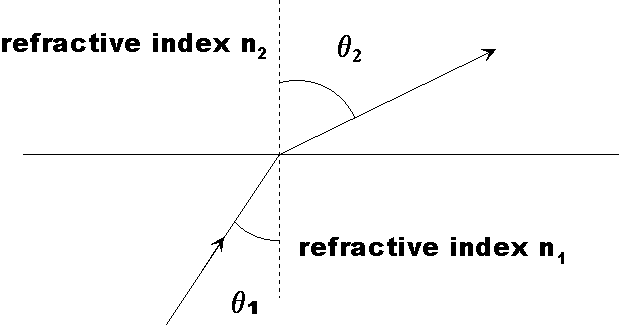
The refractive index of each medium is a number that characterizes the optical density of the medium relative to a vacuum. It is a number that describes how much more slowly light travels in the medium relative to its velocity in a vacuum. If n2 is less than n1 this equation restricts the angles at which a ray of light can get across the boundary. If light is passing from medium 1 to medium 2 and the angle q 1 is greater than the critical angle, then the light can not refract across the boundary (because q 1 can not be greater than 90o). The critical angle is the angle whose sine is n2/n1. When a ray of light strikes a boundary at an angle greater than the critical angle it reflects, and does not cross. Although many devices that we use in real life use this phenomenon, and although many natural optical phenomena depend on its occurrence, we may not be aware of it. A simple example can be given, which involves what a swimmer sees who is underwater and attempting to look upwards through the surface. If such a swimmer looks more or less straight up towards the surface, he or she will be able to see what is above the surface. On the other hand if he or she looks at a sufficiently large angle towards the surface, only a reflection of whatever is below the surface will be seen. Of course, a fish would experience the same effect and can only see over a range of angles near the perpendicular to the surface. This phenomenon is of real practical significance to fisherman, because a fish seeing an attractive lure can not see the rod to which it is attached, nor the fisherman.
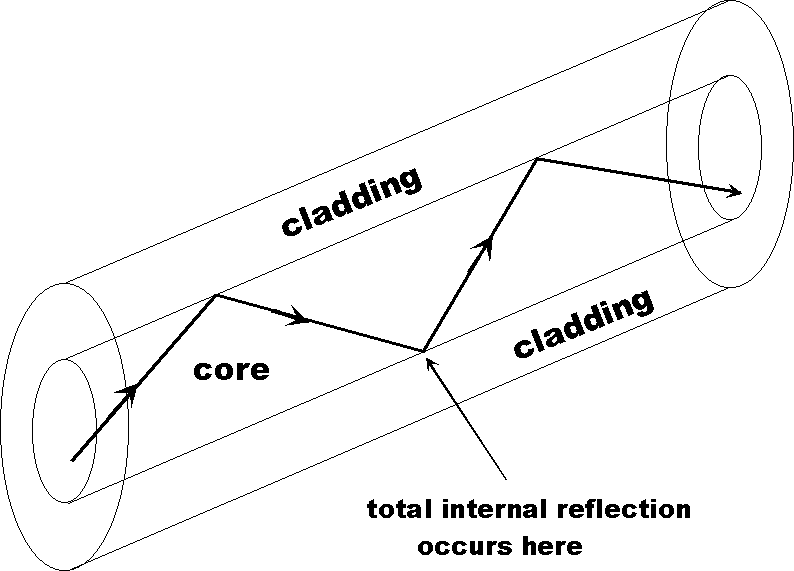
Optical fibers use total internal reflection to keep a light ray trapped within the denser glass of the center of a composite cylindrical glass fiber, the core. It is as if light rays are guided down the core of the fiber in a zigzag path by a succession of total internal reflections at the boundary between the core glass and the less dense glass surrounding it – the cladding, as shown in Fig.(2).
Before discussing the advantages of optical communication along such fibers, we must go back in time to discuss the properties of glass, and how these properties had to be modified to make optical fibers sufficiently transparent to make long distance light transmission along them possible.
A KEY TECHNOLOGICAL DEVELOPMENT - EXTREMELY TRANSPARENT GLASS
Conventional window glass, whether it be soda-lime glass or borosilicate (Pyrex), is not really very transparent. It only appears so because we usually use it in thin sheets – typically a few millimeters thick. If you examine a piece of such sheet glass by viewing it from the narrow edge it is easily seen that the glass has a deep green or brown color when viewed in thicknesses of several centimeters. Even so, glass not much more transparent than this was used, as early as 1957, to make fiber imaging bundles. In these structures, which were primarily developed for medical imaging, a large number of optical fibers are bonded together to make an aligned bundle. An optical image projected onto one end of the bundle is relayed along the bundle, and appears at the far end, where it can be viewed. For viewing distances of a few tens of centimeters, the amount of transmitted light reaching the far end of conventional glass was sufficiently large to allow these imaging bundles to be used for looking into confined spaces, for example inside the body if the fiber was inserted through a body orifice into the esophagus, stomach, or intestine. When these imaging bundles were first used, about 80% of the original light reached the far end of a bundle 1m long. Unfortunately, for a fiber 100 m long, only a ten billionth part of the light reaches the far end – essentially zero. The reduction in transmitted light intensity decreases exponentially with fiber length. We often describe the attenuation properties of the fiber with a measure called the decibel (dB). The attenuation of a length of fiber in dB is determined from the ratio of the input light power (P1) to the output light power at the far end of the fiber (P2) as
Attenuation (dB)= 10 log(P1/P2).
In the example above the attenuation of the fiber is 1000 dB per km (1000 dB/km). Clearly, a fiber with so much attenuation is useless for long distance light transmission. In the early 60s it was quickly realized that unless more transparent glass could be developed, it was merely a dream to contemplate the use of glass fibers to carry information optically over long distances.
Get in Touch
+86-21-3996-3837
alex@changguangchina.com
+86-15216725374